What Makes an Action Potential Rise to 35 Mv? What Makes It Drop Again After This Peak?
6 Activity Potentials
Equally covered in Chapter one, the action potential is a very brief change in the electric potential, which is the difference in accuse between the inside and outside of the cell. During the action potential, the electrical potential across the membrane moves from a negative resting value to a positive value and back.
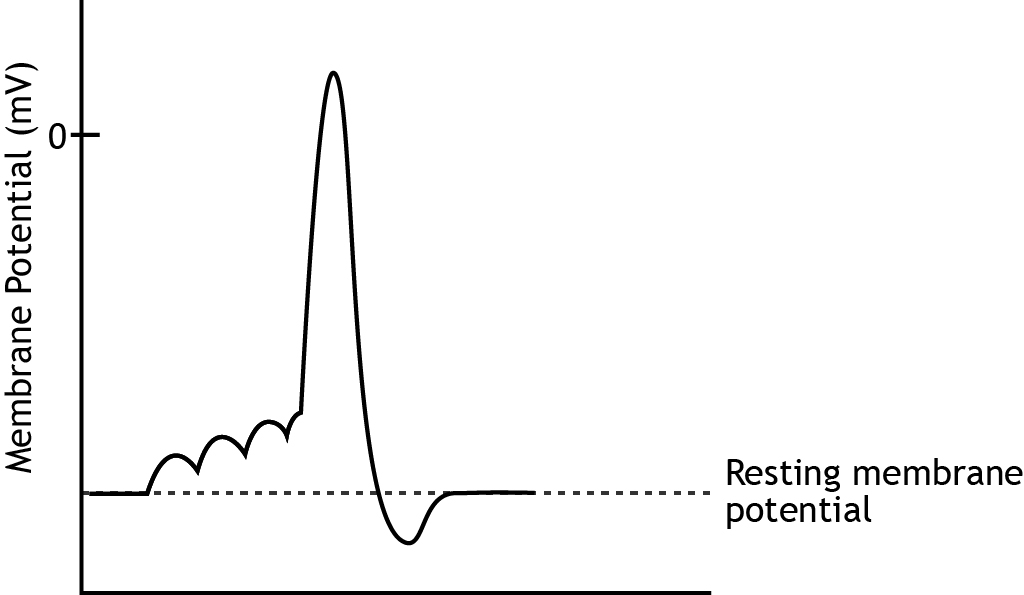
Propagation
The propagation of the action potential from the axon hillock downwardly the axon and to the presynaptic terminal results in release of chemical neurotransmitters that communicate with a postsynaptic neuron.
Blitheness 6.1. The action potential moves downwards the axon kickoff at the axon hillock. The activeness potential moving downwards a myelinated axon will jump from one Node of Ranvier to the adjacent. This saltatory conduction leads to faster propagation speeds than when no myelin in nowadays. When the action potential reaches the synaptic terminal, it causes the release of chemical neurotransmitter. 'Activity Potential Propagation' by Casey Henley is licensed under a Creative Commons Attribution Non-Commercial Share-Alike (CC-BY-NC-SA) iv.0 International License. View static image of blitheness.
Voltage-Gated Ion Channels
The alter in membrane potential during the action potential is a role of ion channels in the membrane. In the previous lessons, nosotros have learned well-nigh the principles of ion movement and take discussed non-gated (leak) channels at rest, as well every bit ion channels involved in the generation of postsynaptic potentials. In this chapter, we volition examine a different type of ion channel: voltage-gated ion channels. For our purposes, these channels are located primarily at the axon hillock, along the axon and at the terminal. They are necessary for the propagation of the activity potential.
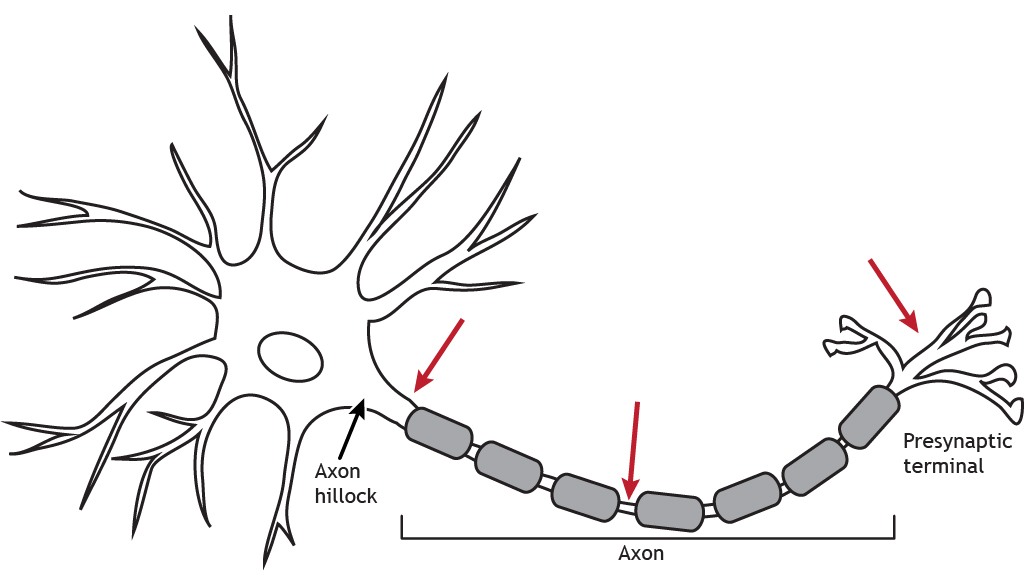
Voltage-gated channels let ions to cantankerous the membrane using the same ion movement principles covered in previous lessons. The chief departure between voltage-gated channels and leak channels are how they are opened or "gated". Voltage-gated channels open when the cell's membrane potential reaches a specific value, called threshold. The neuron reaches threshold after enough EPSPs calculate together.
Blitheness half dozen.ii. Every bit EPSPs summate, a result of ion motion not shown in the blitheness, the cell'southward membrane potential will depolarize. Reaching threshold causes voltage-gated ion channels to open. Once the channels are open, ions will move toward equilibrium. In the animation, sodium ions flow inward. The dotted, bluish channels represent voltage-gated sodium channels; the striped, green channels stand for voltage-gated potassium channels; the solid yellow channels represent chloride channels. 'Voltage-Gated Channel' by Casey Henley is licensed under a Creative Eatables Attribution Non-Commercial Share-Alike (CC-Past-NC-SA) four.0 International License. View static prototype of animation.
The Action Potential
The action potential begins when the cell's membrane potential reaches threshold. One time initiated in a healthy, unmanipulated neuron, the action potential has a consistent structure and is an all-or-nothing effect. It will run through all the phases to completion.
The ascension stage is a rapid depolarization followed by the overshoot, when the membrane potential becomes positive. The falling phase is a rapid repolarization followed past the undershoot, when the membrane potential hyperpolarizes by residue. Finally, the membrane potential volition render to the resting membrane potential.

Rising Phase
The rising phase is caused by the opening of voltage-gated sodium channels. These ion channels are activated once the jail cell'due south membrane potential reaches threshold and open immediately. The electrochemical gradients drive sodium into the cell causing the depolarization.
Animation 6.3. Voltage-gated sodium channels open once the cell's membrane potential reaches threshold. The rapid influx of sodium results in a big depolarization called the rising phase. The dotted, bluish channels represent voltage-gated sodium channels; the striped, greenish channels represent voltage-gated potassium channels; the solid yellow channels stand for chloride channels. 'Rising Phase' by Casey Henley is licensed under a Creative Eatables Attribution Non-Commercial Share-Alike (CC-BY-NC-SA) iv.0 International License. View static image of animation.
Falling Phase
The falling phase of the action potential is acquired by the inactivation of the sodium channels and the opening of the potassium channels. After approximately 1 msec, the sodium channels inactivate. The channel becomes blocked, preventing ion flow. At the aforementioned fourth dimension, the voltage-gated potassium channels open. This allows potassium to rush out of the cell because of the electrochemical gradients, taking its positive charge out of the prison cell, and repolarizing the membrane potential, returning the prison cell'southward membrane potential back near balance.
Similar the voltage-gated sodium channels, the voltage trigger for the potassium channel is when the cell's membrane potential reaches threshold. The difference is that the sodium channels open immediately, whereas the potassium channels open after a filibuster.
Animation 6.4. After approximately ane msec, the voltage-gated sodium channels inactivate, which prevents whatever further ion period into the cell. Although the voltage-gated potassium channels are activated in response to the cell reaching threshold, their opening is delayed and occurs lone with the sodium channel inactivation. This allows an efflux of potassium ions, which causes the repolarization of the falling phase. The dotted, blue channels represent voltage-gated sodium channels; the striped, green channels represent voltage-gated potassium channels; the solid yellow channels represent chloride channels. 'Falling Phase" past Casey Henley is licensed under a Creative Commons Attribution Not-Commercial Share-Alike (CC-BY-NC-SA) 4.0 International License. View static image of animation.
Undershoot
As the membrane potential returns to resting level, the sodium channels will de-inactivate, returning to the closed position, gear up to be opened by a voltage change again. The potassium channels will likewise close, simply they remain open long plenty to cause a hyperpolarizing undershoot as potassium continues to move toward its equilibrium potential of -fourscore mV.
Animation 6.5. Once the cell'due south membrane potential repolarizes, the voltage-gated sodium channels de-inactivate and return to their closed state. The voltage-gated potassium channels remain open long enough for the undershoot to occur as potassium continues to flow out of the cell. The dotted, blueish channels stand for voltage-gated sodium channels; the striped, dark-green channels stand for voltage-gated potassium channels; the solid yellow channels correspond chloride channels. 'Undershoot' by Casey Henley is licensed under a Creative Commons Attribution Non-Commercial Share-Alike (CC-BY-NC-SA) 4.0 International License. View static image of animation.
Render to Rest
Once the voltage-gated channels close, the sodium-potassium pumps will reestablish the proper ionic concentrations needed for the electrochemical gradients. This action along with open leak channels will return the cell to its resting membrane potential.
Animation 6.six. Once the voltage-gated potassium channels close, the sodium-potassium pump will work to re-establish the electrochemical gradients and render the cell to its resting membrane potential. 'Return to Rest' past Casey Henley is licensed under a Creative Commons Attribution Not-Commercial Share-Alike (CC-By-NC-SA) iv.0 International License. View static image of animation.
Refractory Periods
The Absolute Refractory Flow
Each neuron does have a maximum firing rate. And even if the stimulus continues to increase in forcefulness, the neuron cannot burn at a higher frequency. The maximum firing rate of a cell is adamant by the condition of the ion channels in the neuronal membrane during the different phases of the action potential. During the absolute refractory catamenia, a 2nd action potential cannot be fired under any circumstances regardless of the strength of the stimulus. The voltage-gated sodium channels are either open (during the rising phase) or inactivated (during the falling phase).
The Relative Refractory Period
When the cell repolarizes and the voltage-gated sodium channels de-inactivate and render to a airtight state, the cell is again able to burn down another action potential. All the same, during the stop of the falling phase and the during the undershoot, voltage-gated potassium channels are still open. During the undershot, while the neuron is hyperpolarized, a larger-than-normal stimulus is needed to make the cell achieve threshold over again. This segment of the action potential is called the relative refractory menstruation. Action potentials tin exist fired, but a stronger stimulus is needed than when the cell is at rest.
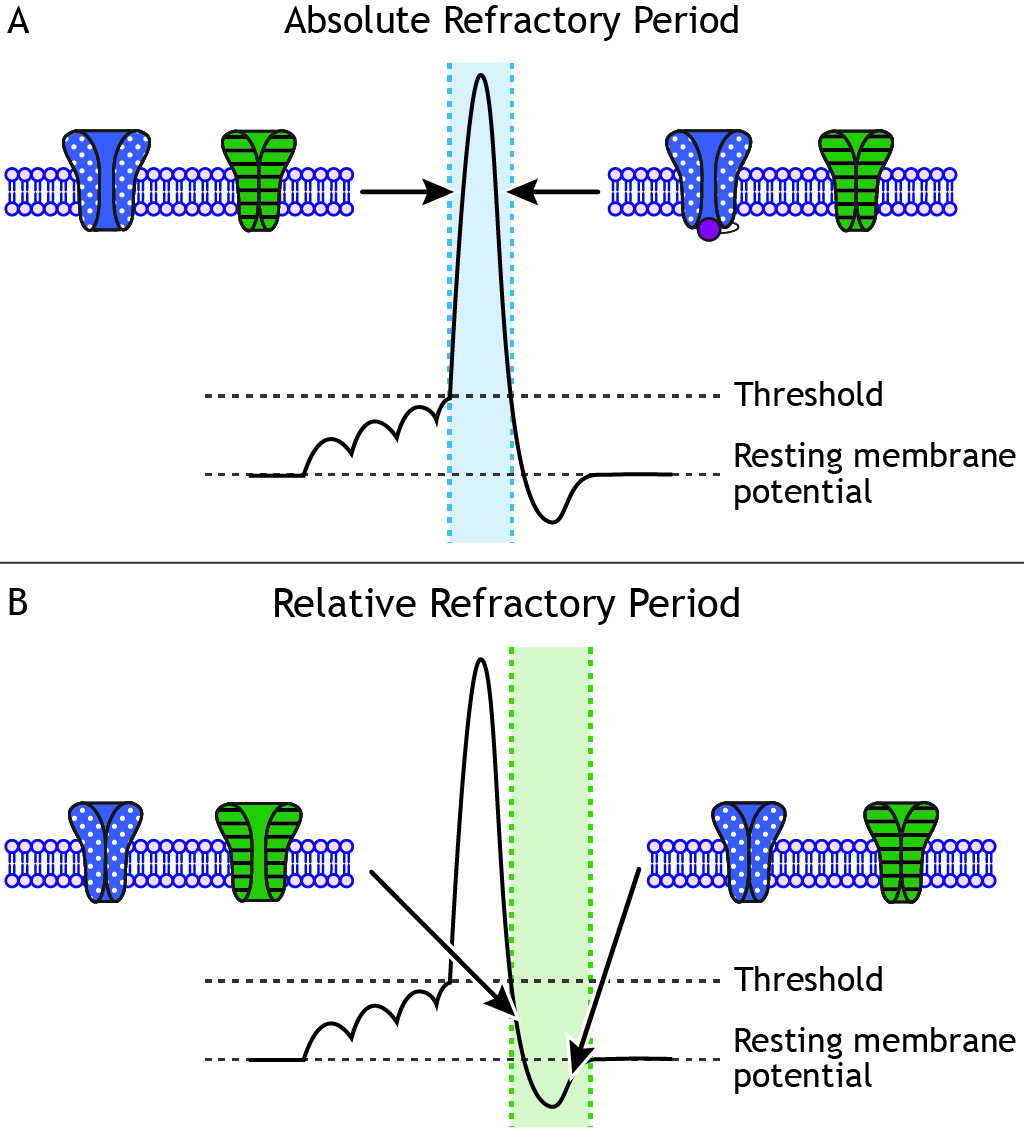
Action Potential Characteristics
For a given cell, all action potentials have the same characteristics; they depolarize to the aforementioned membrane potential value and accept the same amount of time. Nevertheless, unlike neurons may exhibit different activeness potential characteristics. As well, if a neuron has a modify in its environment, like altered extracellular ion concentrations, the shape of the action potential would change due to a modify in the electrochemical gradients. For example, if the external concentration of sodium is decreased, the equilibrium potential of sodium, also equally the strength of the electrochemical gradients will change, which will result in a slower rate of rising and a lower amplitude of the action potential.
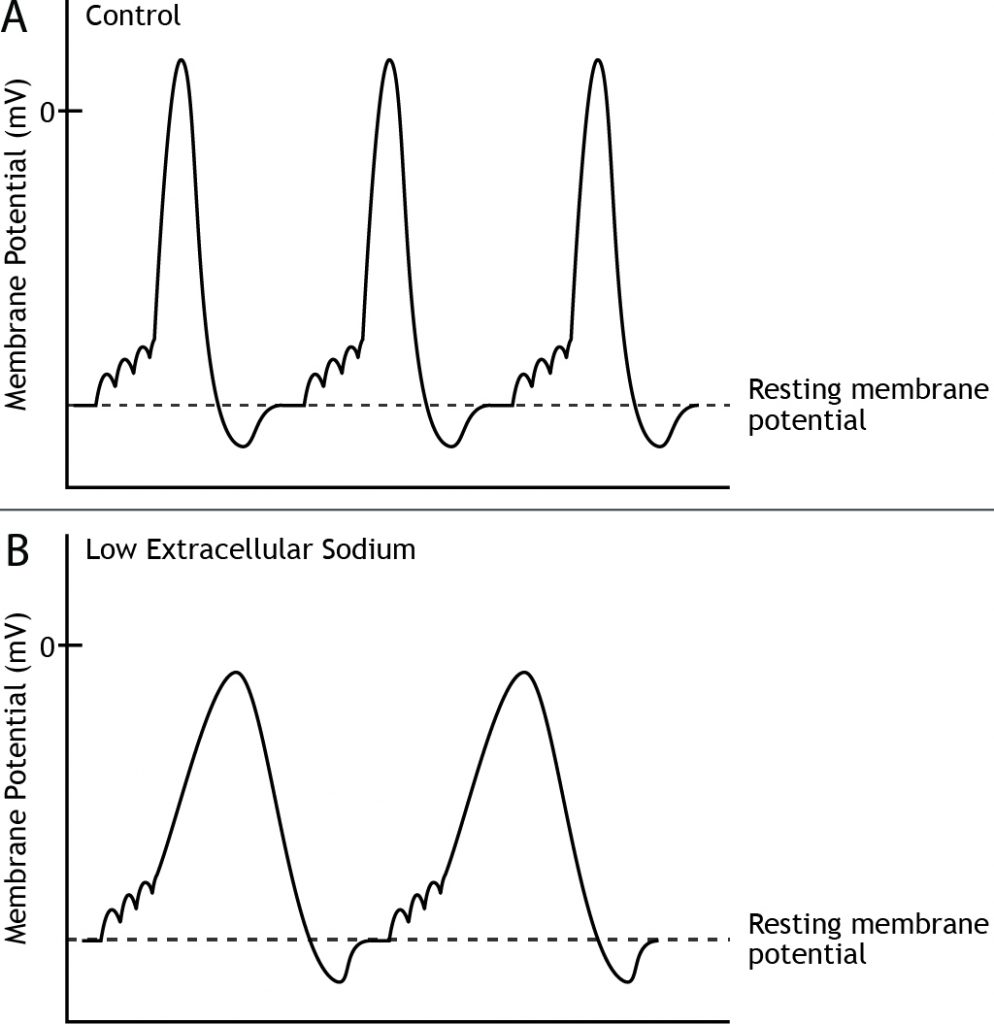
Stimulus Forcefulness
The strength of a stimulus needs to be encoded by the neurons. We need to be able to perceive the difference, for example, betwixt a dim light and a vivid one. The frequency or rate of activity potential firing informs the nervous system of stimulus strength.
Since the height of the action potential is always the same for a given neuron, the forcefulness of the stimulus is determined by the frequency of action potential firing. A weak stimulus would cause fewer action potentials to be fired than a strong stimulus.

Direction of Propagation
The action potential moves downwardly the axon due to the influx of sodium depolarizing nearby segments of axon to threshold.
Animation six.7. A voltage modify that reaches threshold volition cause voltage-gated sodium channels to open up in the axonal membrane. The influx of sodium causes the rising phase of the action potential, just the ion catamenia also depolarizes nearby axon regions. As the depolarization reaches threshold, the action potential moves down the axon. The dotted, blue channels correspond voltage-gated sodium channels; the striped, greenish channels correspond voltage-gated potassium channels. 'Action Potential Movement' by Casey Henley is licensed under a Creative Eatables Attribution Not-Commercial Share-Alike (CC-By-NC-SA) iv.0 International License. View static image of animation.
Action potentials only move in one management, though, from the prison cell trunk to the presynaptic last. The refractory menstruation keeps the activity potential from moving backward down the axon. As the activity potential moves from one Node of Ranvier to the side by side, the inactivated sodium channels in the previous axon segment prevent the membrane from depolarizing over again. Therefore, the action potential can merely movement forward toward axon segments with closed sodium channels fix for ascent stage depolarization.
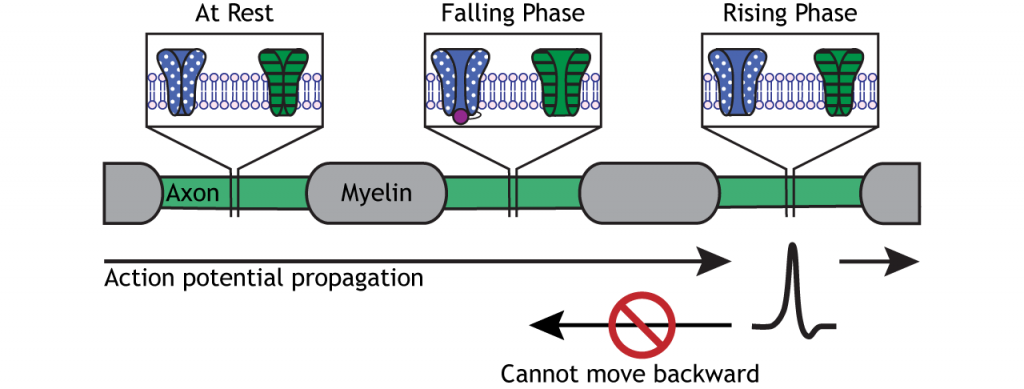
Speed of Propagation
Presence of Myelin
The presence of myelin leads to a pregnant increment in action potential conduction speed compared to an unmyelinated axon. For a myelinated axon, the action potential "jumps" between Nodes of Ranvier in a process called saltatory conduction. The nodes have a high density of voltage-gated channels, and the action potential is able to skip the axon segments covered by the myelin. In an unmyelinated axon, the activeness potential moves in a continuous moving ridge. In additional to the saltatory conduction process, the presence of myelin also insulates the axon, preventing charge loss across the membrane, which also increases speed of the action potential.
Blitheness vi.8. The action potential moves down an unmyelinated axon like a wave, opening voltage-gated channels along the length of the axon. In a myelinated axon, though, the activeness potential is able to skip portions of the axon that are covered past the myelin; the action potential jumps from node to node and travels farther down the axon in the aforementioned amount of time. The dotted, blue channels stand for voltage-gated sodium channels; the striped, green channels represent voltage-gated potassium channels. 'Activeness Potential Speed' by Casey Henley is licensed under a Artistic Commons Attribution Non-Commercial Share-Alike (CC-BY-NC-SA) 4.0 International License. View static epitome of animation.
Diameter of Axon
The bore of the axon also affects speed. The larger the bore of the axon, the faster the propagation of the activity potential downwards the axon. A larger axon leads to less resistance against the flow of ions, and so the sodium ions are able to move more apace to cause the regeneration of the activeness potential in the adjacent axon segment.
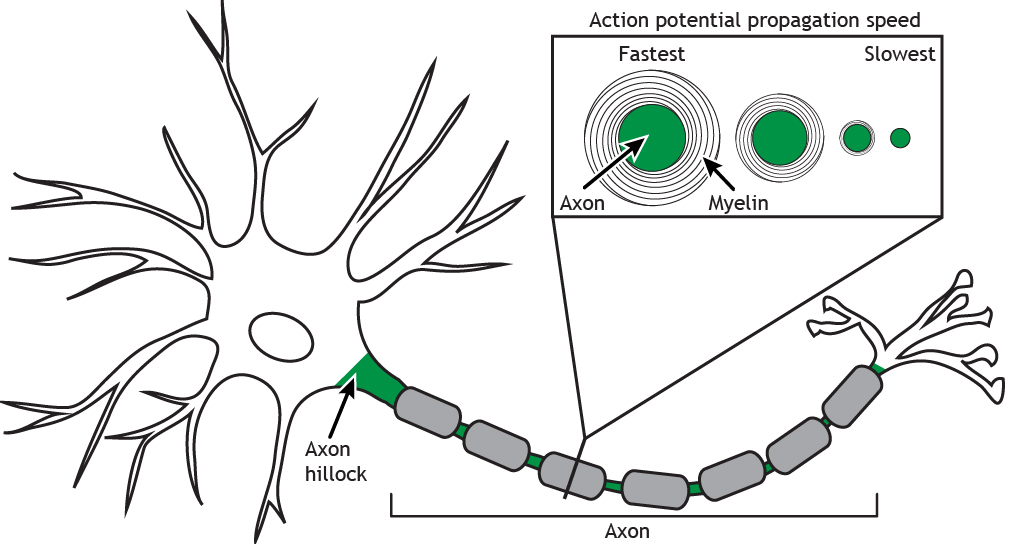
- The voltage-gated ion channels are located along the axon hillock and axon; they open in response to the membrane potential reaching a threshold value
- The rising phase of the action potential is a result of sodium influx
- The falling stage of the activity potential is a result of potassium efflux
- Activeness potentials are all-or-none (postsynaptic potentials are graded)
- Action potential have the same tiptop of depolarization for a given cell under typical conditions
- The neuron cannot fire a second action potential during the absolute refractory phase
- The neuron can fire a 2nd action potential during the relative refractory phase, simply it requires a stronger stimulus than when the neuron is at residual
- Stimulus strength is coded past frequency of activity potential firing
- Activity potential travel in one direction due to the presence of inactivated voltage-gated sodium channels
- Speed of propagation relies on presence and thickness of myelin and diameter of axon
Test Yourself!
Video Lecture
Source: https://openbooks.lib.msu.edu/neuroscience/chapter/action-potentials/